Multi-access edge computing for multi-user VR gaming in 5G and beyond
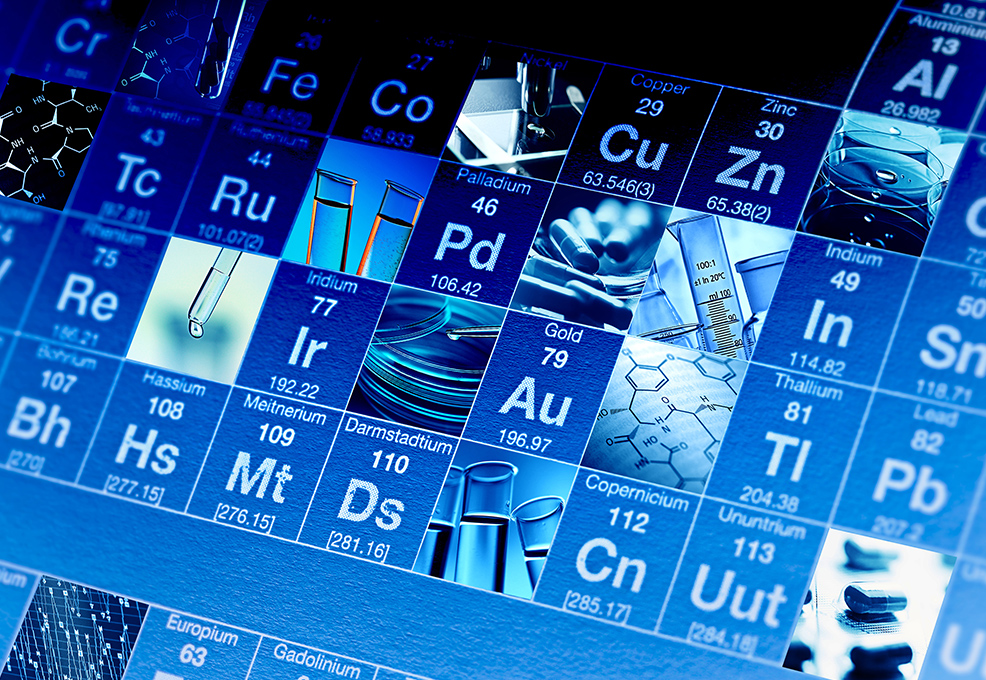
Author(s)
Shih-Kang LinBiography
Dr. Shih-kang Lin is an associate professor of the materials and engineering department, National Cheng Kung University (NCKU). He also serves as the vice director of the research and service headquarters, the vice director of the hierarchical green-energy materials (Hi-GEM) research center, and the chair of the TMS alloy phase committee, USA.
Academy/University/Organization
National Cheng Kung UniversitySource
https://doi.org/10.1016/j.actamat.2019.06.014 https://doi.org/10.1016/j.jallcom.2018.11.290-
TAGS
-
Share this article
You are free to share this article under the Attribution 4.0 International license
- ENGINEERING & TECHNOLOGIES
- Text & Image
- April 21,2020
The history of human beings is the evolution of materials and applications, from the stone age, bronze age, iron age, to the modern intelligent and green-energy era today. Conventionally, the discovery of new materials relies on the experiences and knowledge of materialists and chemists, and involves an extensive number of experiments. The trial-and-error approach is not only a slow process but also very inefficient and costly. Moreover, the known materials are just a small portion of all materials composed of various compositions of elements from the periodic table. With the developments of both materials science and supercomputers, it is now feasible to perform ‘virtual experiments’ using computers. This new approach is known as ‘materials genome’ after a US initiative, which enables efficient material development for national economic growth, defense, and industrial technologies. In this article, the core-knowledge of Prof. Shih-kang Lin’s research group, computational thermodynamics, is briefly introduced, including the applications of ab initio calculations and CALPHAD modeling in designing the emerging energy and structural materials, respectively. The two examples are (1) dopant design for the anode material in lithium-ion batteries and (2) microstructure control and process optimization for free-cutting steels. More accurate and efficient materials design and process optimization with deeper understanding can be achieved. In the future, high-performance-computing, artificial intelligence (AI), and quantum computing are all new tools to speed up computation-aided materials design. The resultant new materials will lead human beings toward a more efficient and sustainable society.
Materials design has been a ‘state-of-the-art’ technology using unique recipe where adding perhaps a minor additive or dopant may dramatically enhance the material properties and/or functionality. The new materials may become new weapons for triumphs or new dominant industrial applications. Conventionally, the discovery of new materials relies on the experiences and knowledge of materialists and chemists and involves an extensive number of experiments. The trial-and-error approach is not only a slow process but also very inefficient and costly. With the developments of both materials science and supercomputers, it is now feasible to perform ‘virtual experiments’ using computers. The following two examples, (1) dopant design for the anode material in lithium-ion batteries and (2) microstructure control and process optimization for free-cutting steels, based on ab initio calculations and CALPHAD modeling, respectively, are briefly introduced, highlighting computational thermodynamics as a powerful tool for material development for both energy and structural materials.
(1) Dopant design for the anode material in lithium-ion batteries
Lithium titanate defect spinel (Li4Ti5O12, LTO) is an anode material in lithium-ion batteries with a long cycling life and high safety; however, its intrinsic electrical conductance needs to be improved by proper dopants. Based on ab initio calculations, sodium (Na), potassium (K), magnesium (Mg), calcium (Ca), strontium (Sr), aluminum (Al), and gallium (Ga) were systematically investigated as the dopant in terms of stability in LTO, electrical conductivity, and electrochemical kinetics. As shown in Fig. 1, Mg can stably substitute Li at the tetrahedral site (8a), providing electrons to reduce the neighboring Ti ions. Consequently, the concentration of the Ti3+/Ti4+ pairs is increased to facilitate more electron hopping. Therefore, when the doping level of Mg exceeds the threshold (x ~ 0.05), the electrical conductivity of LTO dramatically increases from ca. 10-8 S/cm to ca. 10-2 S/cm. The great enhancement in electrical conductance promotes the rate capacity of the anode materials. The 10C high-rate charging (fully charged in 6 mins.) capacity is increased by 33%.
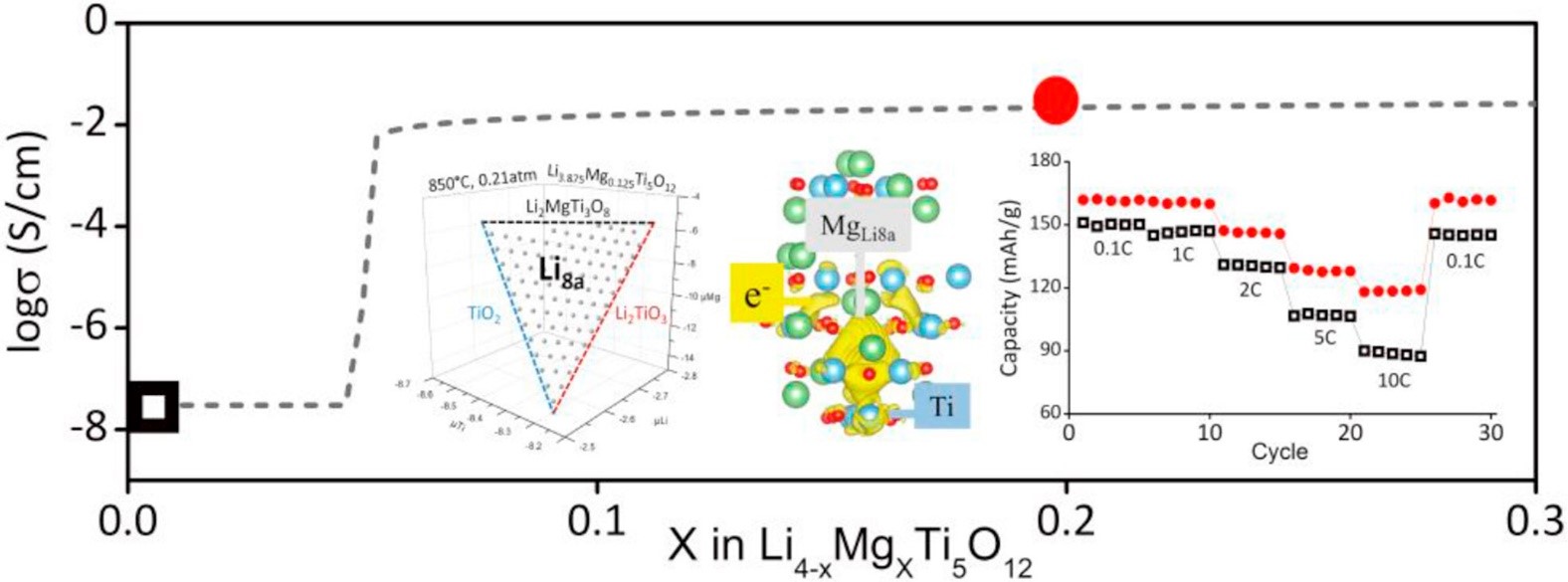
Figure 1. Effect of Mg doping in Li4Ti5O12 (LTO) as an anode material in lithium-ion batteries on phase stability, charge distribution, electrical conductivity, and rate capability (Acta Mat., 2019).
(2) Microstructure control and process optimization for free-cutting steels
Efficiency of cutting is demanded in the steel industry. The manganese sulfide (MnS) inclusions are stress raisers in free-cutting steels, which lower the cutting resistance and hence improve the manufacturing efficiency. The morphology of MnS critically determines its property as a stress raiser in the steels, so its formation and morphology during casting must be well controlled. Based on CALPHAD thermodynamic modeling, the effects of hydrogen (H), boron (B), carbon (C), nitrogen (N), oxygen (O), aluminum (Al), silicon (Si), phosphor (P), argon (Ar), vanadium (V), chromium (Cr), cobalt (Co), nickel (Ni), copper (Cu), arsenide (As), zirconium (Zr), niobium (Nb), molybdenum (Mo), tin (Sn), titanium (Ta), tungsten (W) as the alloying elements upon the solidification of MnS and microstructure were systematically investigated. As shown in Fig. 2, O was found to be a super-strong stabilizer for monotectic reaction during solidification, which enables the desirable round-shaped MnS. The computational materials screening provides an effective and efficient guideline for MnS morphology control in free-cutting steels.
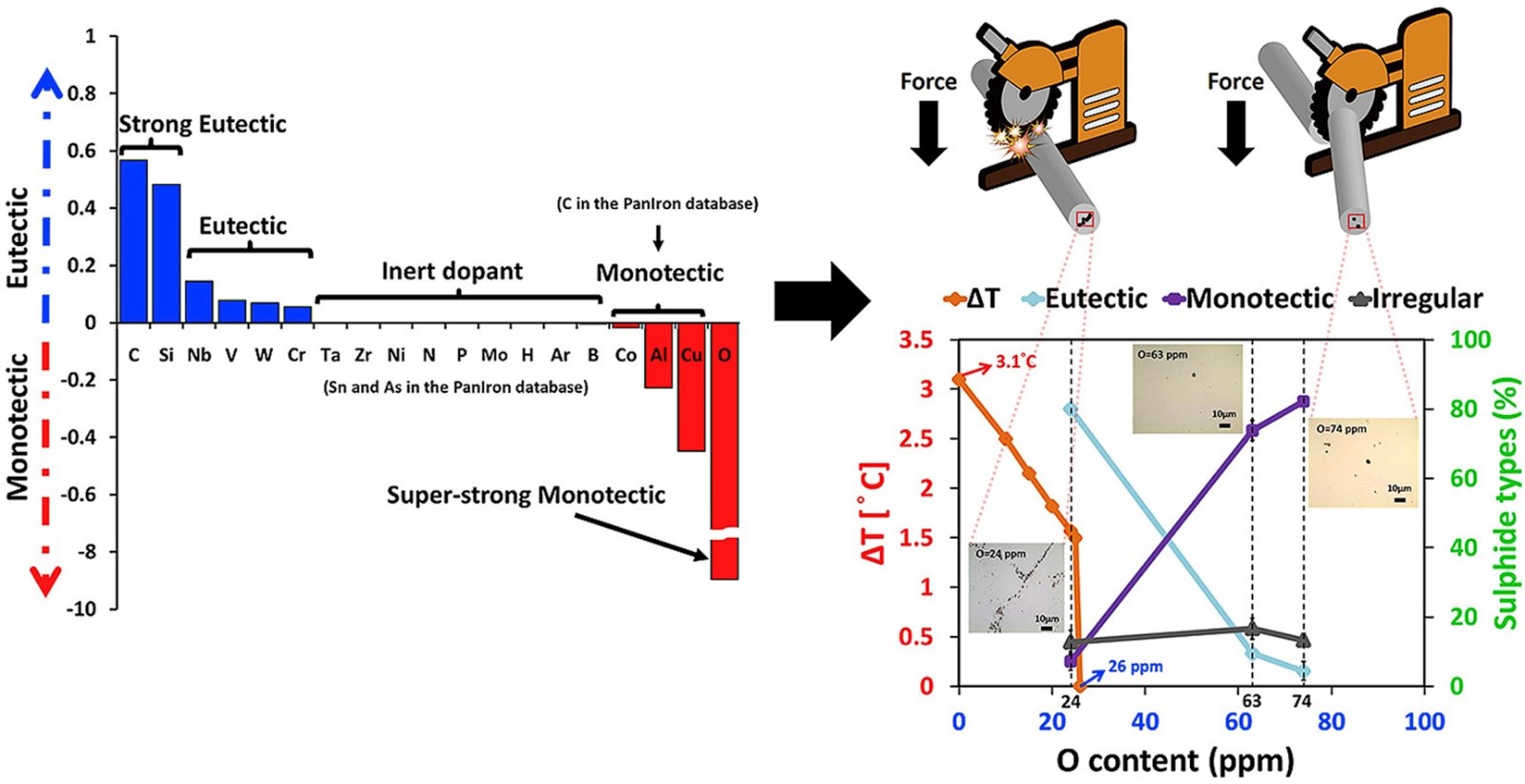
Figure 2. The effect of various alloying elements on the MnS solidification reactions (eutectic or monotectic) and the 1600°C high-temperature validations of O as a super-strong monotectic MnS stabilizer (J. Alloy Compd., 2019)
STAY CONNECTED. SUBSCRIBE TO OUR NEWSLETTER.
Add your information below to receive daily updates.